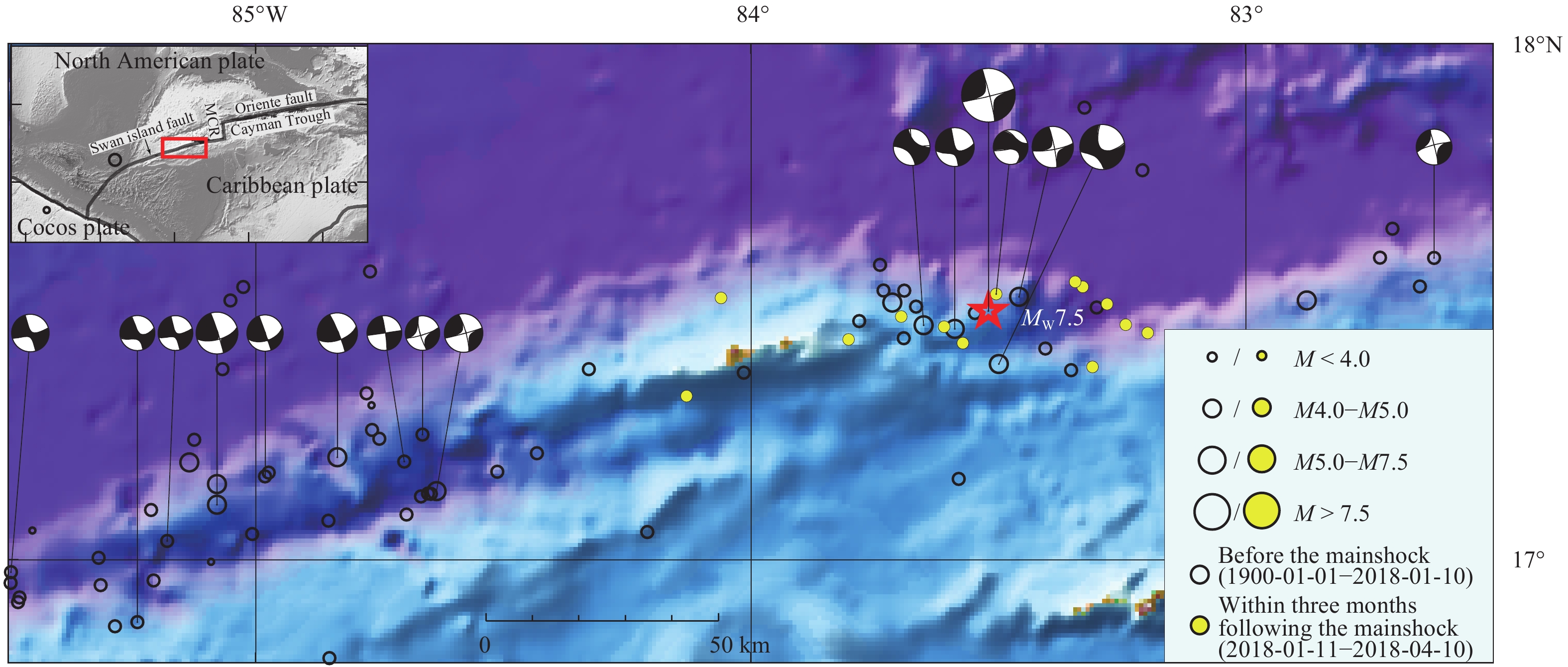
Citation: | Chuang Cheng, Dun Wang (2020). Imaging the rupture process of the 10 January 2018 MW7.5 Swan island, Honduras earthquake. Earthq Sci 33(4): 194-200. DOI: 10.29382/eqs-2020-0194-03 |
On 10 January 2018 (UTC), a MW7.5 earthquake occurred in the east of Great Swan island, Honduras (location 17.483°N, 83.520°W, depth 19.0 km), according to the U.S. Geological Survey (USGS). Nine other earthquakes of M6 or larger have occurred within 400 km of the January 10, 2018 event over the preceding century.
Previous strong earthquakes in this region include a destructive M7.5 Guatemala earthquake on 4 February 1976, which resulted in more than 23,000 fatalities and occurred 650 km west-southwest of the 2018 event. In May 2009, a M7.3 earthquake occurred approximately 300 km west of the 2018 event. The 2009 earthquake (which was much closer to land than the 2018 event) resulted in 7 fatalities, 40 injuries and 130 buildings being damaged or destroyed (according to the USGS).
The dominant tectonic element in the source areas is the Cayman Trough, an 80-km-wide, 1000-km-long and 5-km-deep ocean basin formed at the north-south-trending Mid-Cayman spreading center (Brinka et al., 2002; Mann et al., 1991) (Figure 1). The Cayman Trough originated as a pull-apart basin in early to middle Eocene time and is currently spreading along the Mid-Cayman spreading center at about 15 mm/a (Mann et al., 1991; Rosencrantz and Sclater, 1986). The Cayman Trough is a region of oceanic crust, and is the transform plate boundary between the North American and Caribbean plates. It was formed at the Mid-Cayman Rise (MCR) oceanic spreading center. The linear ridges and rough topography of the central Cayman Trough are typical of active spreading centers (Brinka et al., 2002). The trough is bounded on the south by the Nicaragua Rise, a shallow submerged platform and on the north by the Cayman Ridge, a narrow sliver that was probably part of the Nicaragua Rise before the opening of the trough (Brinka et al., 2002).
The south wall of the Cayman Trough includes and is defined by active strike-slip (transform) faulting, to the east of the spreading axis as well as to the west (Rosencrantz and Mann, 1991). The Cayman Trough contains a ~100-km-long slow-spreading ridge between two offset transform faults, the Swan islands fault and the Oriente fault (Brinka et al., 2002; Holcombe et al., 1973). To the west of the MCR, the Cayman Trough is bounded by the left-lateral strike-slip Swan island fault. To the east of the MCR, the Cayman Trough is bounded on the north by the Oriente fault. On the basis of bathymetric and seismic information, Holcombe et al. (1973) showed that the rugged topography central to the Trough was symmetrical to a north-south trending, deep, flat-floored valley at longitude 81°40’W and that this valley marked the location of a spreading center connecting the two transform faults (Rosencrantz and Sclater, 1986). The western fault, known as the Swan islands transform fault, connects the southern end of the Cayman spreading axis with the Motagua fault in Central America (Holcombe et al., 1973; Rosencrantz and Mann, 1991). The three earthquakes mentioned above occurred on the east segment of the left-lateral strike-slip Swan fault, which is moving with a speed of 18.4 mm/a (Rogers and Mann, 2007).
This earthquake is an erect left-lateral strike-slip faulting, with a magnitude of MW7.5 and a focal depth of about 10 km (according to the USGS). The rupture does not show obvious directivity and the slip distribution on the fault plane is rather concentrated (Wang et al., 2018). The ruptured slip is compact, with a maximum slip of 1.9 m.
Here we apply a back-projection method to trace the rupture migration of the 2018 MW7.5 earthquake using seismic data recorded in Alaska, US. The results show that at least three faults were ruptured during the earthquake. The rupture speed of the ruptured fault with large energy radiation and longest source length, shows a fast rupture speed of ~5 km/s, which is faster than local shear wave velocity of ~4 km/s based on the Crust 1.0 (http://igppweb.ucsd.edu/~gabi/rem.html, accessed February 2020).
Follow Wang et al. (2016), we back-project the source process of the 2018 Honduras earthquake, and estimate the rupture speed. Since the success of exploring source extent and duration of the 2004 M9.0 Sumatra earthquake (Ishii et al., 2005; Kruger and Ohrnberger, 2005), the back-projection method has been widely used in probing source characteristics and resolving complex source patterns (Meng et al., 2011; Wang et al., 2012; Yao et al., 2012; Fan and Shearer, 2017).
We utilize data recorded at 330 seismic stations in Alaska to back-project the source process of the 2018 MW7.5 Honduras earthquake. The seismic stations were located at distances of 45–78 degrees to the epicenter, with azimuths of 321–340 degrees (Figure 2a). The observed waveforms are aligned with P arrivals (Figure 2b), showing high similarities among each other. The high similarities of the observed waveforms suggest fewer site effects among stations, and ensure high accuracy of back-projection results. Since all the waveforms are similar, the differences in travel times caused by source migrations will be accurately determined by comparing the stacked energies of the tested grids in the source region. This is the key why regional arrays usually get better results than those determined by global arrays in back-projection. Due to large differences of structure heterogeneity in ray paths and directivity effect, global observations show considerable differences at high-frequency bands (say higher than 0.5 Hz).
The observed waveforms are first band pass filtered in the band of 0.01–0.2 Hz using a two-pole Butterworth filter. We cross correlate a time window of 20 s (starting from the P onset) with a offset of 5 s, and apply these obtained station corrections to the waveforms. Then we cross correlate a second time window (6 s) for the higher frequency bands of 0.5 Hz to 2.0 Hz with a small offset (0.5 s). We estimate the station corrections in two steps to avoid cycle slip in the high band passed waveforms that are utilized in back-projection. We set up a grid of 100×40 points at a focal depth of 19 km according to the USGS, and use a shifting time window of 10 s that is offset by 1 s. The velocity structure model used in calculating travel times between grid points and seismic stations is IASPEI 1991 (Kennett and Engdahl, 1991).
Our results show that the earthquake initiated from the Swan island fault, then unilaterally propagated to the east (Figure 3). About 10 s after the origin time, the rupture propagated along an SW fault that is obliquely crossing the general striking direction of the Swan island fault for another 10 s. 20 s after the origin time, the rupture migrated to the east with a duration of ~20 s. 40 s after the origin time, the stacked energies decreased, and the locations became scattered, suggesting that the end of the main rupture and/or lower resolution in back-projection in these time windows (Figure 4a). The total source duration is around 40 s (Figure 4b).
Because the rupture was not simply over a straight fault, the rupture speed of this earthquake cannot be estimated by a straight distance to the epicenter for the entire process. At least three faults were ruptured during this earthquake. Thus, we set three reference points at the starting place of three ruptured faults, to better estimate the rupture speeds of the earthquake at the three stages (Figure 4c).
In the first 10 s, the rupture speed is as low as 1 km/s, similar to the initial rupture speeds of many other large earthquakes (Wang et al., 2016). In the second stage, that is, 10–20 s after the origin time, the rupture speed is around 2–3 km/s depending on assumed fault orientation and rupture starting points (Figure 4d). Because of the relatively short length of the rupture fault (~20 km) in this stage, and considering a 10–20 km uncertainty in locations of the local maximums, the uncertainty of the estimated rupture speed in this stage is relatively large. About 20 s after the origin time, the rupture propagated to the west with a fast rupture speed of 5.3 km/s. The radiated energy reached a peak at this stage. Therefore, the observed rupture locations are the most trustable. Considering that the shear wave velocity is 4.05 km/s at the focal depth (19 km) according to the Crust 1.0 (Laske et al., 2013), the rupture speed is faster than local shear wave speed, supporting a previous study that suggests that supershear rupture is common in large strike-slip earthquakes (Wang et al., 2016).
The complicated rupture pattern is evidenced by the global bathymetry and topography of SRTM 15+ (Tozer et al., 2019) and the original satellite scenes of Google Earth. In both the SRTM 15+ and the Google Earth, several NW faults that are obliquely crossing the Swan island fault are obvious in the source region. The SW fault in stage 3 is also well outlined by the bathymetric offsets (Figure 5). Although we couldn’t verify the ruptured faults on site, the bathymetric data supports the complicated rupture pattern we determined from the back-projection.
Our results show a rupture model for the 2018 MW 7.5 Honduras earthquake with at least three ruptured faults. The stages 1 and 3 are along the general strike direction of the Swan island fault, and the stage 2 is obliquely crossing the Swan island fault. The strike direction of the stage 2 is similar to the layout of the aftershocks that occurred in the east of the mainshock epicenter. As more seismic stations have been deployed and new methods are developed, such complexities in large earthquakes are more and more frequently observed in recent cases, such as the 2012 MW8.6 Off-Sumatra (Satriano et al., 2012; Wang et al., 2012; Yue et al., 2012; Wei et al., 2013) and the 2016 MW7.8 New Zealand earthquakes (Hamling et al., 2017; Kaiser et al., 2017; Zhang et al., 2017; Wang et al., 2018). Even for a MW5.5 earthquake, with dense seismic observations, we observed rupture over several faults during the earthquake sequence (Li et al., 2019).
There were few oceanic strike-slip earthquakes identified as supershear rupture speed, mostly due to the space distributions of seismic observations in ocean. Only the 2012 MW8.6 and MW8.2 Off-Sumatra earthquakes and the 5 January 2013 Craig, Alaska earthquake (MW7.5) showed evidences of supershear ruptures (Wang et al., 2012; Meng et al., 2012; Yue et al., 2013). The 2012 MW8.6 Indo-Australia intraplate oceanic earthquake was the largest strike-slip event yet observed. The rupture process of the Indo-Australia event was very complex, involving rupture of no less than four segments. The 5 January 2013 MW7.5 Craig, Alaska earthquake was the first oceanic interplate event reported involved supershear rupture, and it was the first offshore event for which multiple data set independently indicated supershear rupture. Since those earthquakes were with large magnitudes and were ruptured in relatively long faults, resolving their rupture speeds is relatively easier. With more and more dense regional seismic arrays and technological progress, we might be able to reveal more details of rupture patterns of oceanic earthquakes with lower magnitudes in the near future.
More detailed rupture patterns help better estimate rupture parameters, such as rupture speed and source extent. Rupture speed, as a key parameter that controls earthquake strong motion, is difficult to estimate due to lack of near field observations. However, knowing rupture speed is important in rupture dynamics and earthquake engineering. When rupture speed is as slow as several kilometers per hour (slow earthquakes), they don’t cause any shaking damages (Figure 6). Tsunami earthquakes usually have rupture speeds of ~1 km/s, causing small to moderate shaking damages. When the rupture speed reaches a higher level, saying faster than local shear wave velocity, it will generate Mach waves, creating widespread and strong damages if there is a large population around the source area. Such supershear ruptures were only observed in long and straight faults such as the Kunlun fault and the Denali fault, partly because long distance and simple fault pattern make the rupture easily estimated. For strike-slip earthquakes with complex rupture patterns, correctly estimating rupture speed remains challenging. Therefore, many rupture speeds of moderate to large earthquakes derived from simple fault patterns exist large uncertainties, and worth further investigations.
We analyze the rupture process of the 1 January 2018 MW7.5 Honduras earthquake by back-projecting the seismic data recorded at Alaska stations. Our results show that at least three faults were ruptured during the earthquake. The earthquake started from an SW striking fault in the first 10 s, then jumped to a NW striking fault for another 10 s. 20 s after the origin time, the rupture propagated along an SW striking fault for 20 s. The rupture speeds for the first two stages were 1 km/s and 1–3 km/s, respectively. The longest segment, stage 3, showed a clear and fast supershear rupture speed, as fast as 5.3 km/s. There are many oceanic strike-slip earthquakes, only a few of them have been verified as ruptured supershear. This study, together with recent literature, suggests that supershear rupture may commonly occur in strike-slip earthquakes.
This work is supported by the National Key R&D Program of China (No. 2018YFC0603500), Programme on Global Change and Air-Sea Interaction (GASI-GEOGE-02), NSFC (Nos. 41474050, 41425012, 41874062 and 41922025), 111 project (No. BP0719022), and the Fundamental Research Funds for the Central Universities, China University of Geosciences (Wuhan) CUG170602 (D.W.). The moment tensor solutions of the mainshock are available at U.S. Geological Survey (USGS, https://earthquake.usgs.gov/earthquakes/eventpage/us1000c2zy/executive, accessed February 2020), Global Centroid Moment Tensor (GCMT, https://www.globalcmt.org/CMTsearch.html, accessed February 2020). Figures are generated using the Generic Mapping Tools (Wessel et al., 2013).
Brinka UST, Colemanb DF and Dillona WP (2002) The nature of the crust under Cayman Trough from gravity. Marine and Petroleum Geology 19(8): 971–987 doi: 10.1016/S0264-8172(02)00132-0
|
Fan W and Shearer PM (2017) Investigation of Backprojection Uncertainties With M6 Earthquakes. J Geophys Res: Solid Earth 122(10): 7966– 7986
|
Hamling IJ, Hreinsdóttir S, Clark K, Elliott J, Liang C, Fielding E, Litchfield N, Villamor P, Wallace L and Wright TJ (2017) Complex multifault rupture during the 2016 MW 7.8 Kaikōura earthquake, New Zealand. Science 356(6334): eaam7194 doi: 10.1126/science.aam7194
|
Holcombe TL, Vogt PR, Matthews JE and Murchison RR (1973) Evidence for sea-floor spreading in the Cayman Trough. Earth Planet Sci Lett 20(3): 357–371 doi: 10.1016/0012-821X(73)90011-3
|
Ishii M, Shearer PM, Houston H and Vidale JE (2005) Extent, duration and speed of the 2004 Sumatra-Andaman earthquake imaged by the Hi-Net array. Nature 435(7044): 933–936 doi: 10.1038/nature03675
|
Kaiser A, Balfour N, Fry B, Holden C, Litchfield N, Gerstenberger M, D’Anastasio E, Horspool N, Mcverry G and Ristau J (2017) The 2016 Kaikōura, New Zealand, earthquake: preliminary seismological report. Seismol Res Lett 88(3): 727–739 doi: 10.1785/0220170018
|
Kennett BLN and Engdahl ER (1991) Traveltimes for global earthquake location and phase identification. Geophys J R Astr Soc 105(2): 429–465 doi: 10.1111/j.1365-246X.1991.tb06724.x
|
Kruger F and Ohrnberger M (2005) Tracking the rupture of the MW = 9.3 Sumatra earthquake over 1,150 km at teleseismic distance. Nature 435(7044): 937–939 doi: 10.1038/nature03696
|
Laske G, Masters G, Ma Z and Pasyanos M (2013) Update on CRUST1. 0—A 1-degree global model of Earth’s crust. EGU General Assembly Conference Abstracts 15: 2658
|
Li Y, Wang D, Xu S, Fang L, Cheng Y, Luo G and Mori J (2019) Thrust and Conjugate Strike-Slip Faults in the 17 June 2018 MJMA 6.1 (MW 5.5) Osaka, Japan, Earthquake Sequence. Seismol Res Lett 90(6): 2132 – 2141 doi: 10.1785/0220190122
|
Mann P, Tyburski SA and Rosencrantz E (1991) Neogene development of the Swan Islands restraining-bend complex, Caribbean Sea. Geology 19(8): 823–826 doi: 10.1130/0091-7613(1991)019<0823:NDOTSI>2.3.CO;2
|
Meng L, Ampuero JP, Stock J, Duputel Z, Luo Y and Tsai VC (2012) Earthquake in a maze: compressional rupture branching during the 2012 MW8.6 sumatra earthquake. Science 337(6095): 724–726
|
Meng L, Inbal A and Ampuero JP (2011) A window into the complexity of the dynamic rupture of the 2011 MW 9 Tohoku-Oki earthquake. Geophys Res Lett 38(7): L00G07
|
Rosencrantz E and Mann P (1991) SeaMARC II mapping of transform faults in the Cayman Trough, Caribbean Sea. Geology 19(7): 690–693 doi: 10.1130/0091-7613(1991)019<0690:SIMOTF>2.3.CO;2
|
Rogers RD and Mann P (2007) Transtensional deformation of the western Caribbean-Northern America plate boundary zone. In: Mann P (ed) Geologic and Tectonic development of the Caribbean plate in northern Central America. Geological Society of America Special Paper, pp 37–46 doi: 10.1130/2007.2428(03)
|
Rosencrantz E and Sclater JG (1986) Depth and age in the Cayman Trough. Earth Planet Sci Lett 79(1-2): 133–144 doi: 10.1016/0012-821X(86)90046-4
|
Satriano C, Kiraly E, Bernard P and Vilotte JP (2012) The 2012 MW 8.6 Sumatra earthquake: Evidence of westward sequential seismic ruptures associated to the reactivation of a N-S ocean fabric. Geophys Res Lett 39(15): L15302
|
Tozer B, Sandwell D, Smith W, Olson C, Beale J and Wessel P (2019) Global bathymetry and topography at 15 arc sec: SRTM15+. Earth and Space Science 6(10): 1847 – 1864 doi: 10.1029/2019EA000658
|
Wang D, Chen Y, Qi W and Mori J (2018) Complex rupture of the 13 November 2016 MW7.8 Kaikoura, New Zealand earthquake: Comparison of high-frequency and low-frequency observations. Tectonophysics 733: 100–107
|
Wang D, Mori J and Koketsu K (2016) Fast rupture propagation for large strike-slip earthquakes. Earth Planet Sci Lett 440: 115–126 doi: 10.1016/j.jpgl.2016.02.022
|
Wang D, Mori J and Uchide T (2012) Supershear rupture on multiple faults for the MW8.6 Off Northern Sumatra, Indonesia earthquake of April 11, 2012. Geophys Res Lett 39(21): L21307
|
Wang WM, Hao JL and Yao ZX (2018) Preliminary results for the rupture process of Jan.10, 2018, MW7.6 earthquake at east of Great Swan Island, Honduras. Earth Planet Phys 2(01): 88–89
|
Wei S, Helmberger D and Avouac JP (2013) Modeling the 2012 Wharton basin earthquakes off-Sumatra: Complete lithospheric failure. J Geophys Res: Solid Earth 118(7): 3592 – 3609 doi: 10.1002/jgrb.50267
|
Wessel P, Smith W, Scharroo R, Luis J and Wobbe F (2013) Generic mapping tools: improved version released. Eos Transactions American Geophysical Union 94(45): 409–410
|
Yao H, Shearer PM and Gerstoft P (2012) Subevent location and rupture imaging using iterative backprojection for the 2011 Tohoku MW9.0 earthquake. Geophys J Int 190(2): 1152 – 1168 doi: 10.1111/j.1365-246X.2012.05541.x
|
Yue H, Lay T and Koper KD (2012) En échelon and orthogonal fault ruptures of the 11 April 2012 great intraplate earthquakes. Nature 490(7419): 245–249 doi: 10.1038/nature11492
|
Yue H, Lay T, Schwartz SY, Rivera L, Protti M, Dixon TH, Owen S and Newman AV (2013) The 5 september 2012 nicoya, costa rica MW7.6 earthquake rupture process from joint inversion of high-rate gps, strong-motion, and teleseismic P wave data and its relationship to adjacent plate boundary interface properties. J Geophys Res: Solid Earth 118(10): 5453– 5466
|
Zhang H, Koper KD, Pankow K and Ge Z (2017) Imaging the 2016 MW7.8 Kaikoura, New Zealand, earthquake with teleseismic P waves: A cascading rupture across multiple faults. Geophys Res Lett 44(10): 4790 – 4798 doi: 10.1002/2017GL073461
|
1. | Gamboa-Canté, C., Arroyo-Solórzano, M., Benito, B. et al. Seismicity in Central America (1520–2020) and Earthquake catalog compilation for seismic hazard assessments. Bulletin of Earthquake Engineering, 2024. DOI:10.1007/s10518-024-02059-9 | |
2. | Cheng, C., Wang, D., Yao, Q. et al. The 2021 Mw 7.3 Madoi, China Earthquake: Transient Supershear Ruptures on a Presumed Immature Strike-Slip Fault. Journal of Geophysical Research: Solid Earth, 2023, 128(2): e2022JB024641. DOI:10.1029/2022JB024641 | |
3. | Zhu, S., Cui, Z. Why are there so few supershear rupture earthquakes in real-world nature? | [为什么自然界中超剪切破裂的地震是如此之少?]. Acta Geophysica Sinica, 2022, 65(1): 51-66. DOI:10.6038/cjg2022P0828 | |
4. | Guzmán-Speziale, M.. The triple junction of the North America, Cocos, and Caribbean plates. What we know, what we don’t. Revista Mexicana de Ciencias Geologicas, 2022, 39(2): 190-205. DOI:10.22201/cgeo.20072902e.2022.2.1666 |